|
 |
VISION AND OPTIC REFLEXES
The eyes are truly remarkable organs. Their light-sensitive
retinas are able to convert light rays from objects in the
visual field to impulses on the optic nerves, which ultimately
give rise to images in the visual cortex of the brain. These
images are usually sharp and clear because of the focusing power
of the lens. As if the ability to perceive images isn't enough,
the eyes are also able to function under widely varying
conditions. For example, they are able to adjust to viewing near
and far objects, large and small objects, moving and stationary
objects as well as objects in bright daylight and under the poor
light conditions of night vision. A number of optic reflexes
enable the eyes to make these adjustments.
THE EYE AND
THE PATH OF LIGHT
Light from an object in the visual field must pass through the
cornea, the aqueous humor, the pupillary aperture, the lens, and
the vitreous humor before reaching the light-sensitive retina
(Fig-1). When we look directly at an object, the light rays
from that object are focused on an especially sensitive area of
the retina, the
fovea.
Items in the peripheral visual field are focused on the
remainder of the retina. Both kinds of photoreceptors (rods and
cones) are located throughout the peripheral retina, while the
fovea contains only cones. The
optic disk.
formed by the confluence of the optic nerve fibers from the
nasal (medial) and temporal (lateral) portions of the retina, is
devoid of any photoreceptors and is called the
blind spot.
Refractive Power
and Accommodation of the Lens
Light rays entering the eyes are selectively bent as they pass
through the cornea, aqueous humor, lens, and vitreous humor on
their way to the retina. While each of these contributes
somewhat to bending the entering light rays, it is nevertheless
the lens which is responsible for bending the rays sufficiently
to focus them on the retina since it is the only refractive
surface which can change its light bending (refractive)
capability. The other three are all fixed values which give rise
to the same amount of bending whether the eye is focused for
distant
or near vision. |
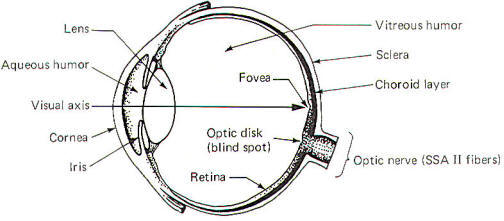 |
Fig-1 |
The refractive power L
of the lens is measured in diopters and is equal to
the reciprocal of the focal length F expressed in meters.
The natural tendency for the lens is to assume a curved shape,
giving it a high refractive power and dioptric strength. Now
when the eye is focused for distant vision (any distance greater
than about 20 ft),
the lens is pulled relatively flat and has only minimal
refractive power. Nevertheless, even in this condition it does
bend light and has a refractive power equal to 18 diopters. When
the eye focuses on objects closer than 20 ft,
the refractive power of the lens increases in order to focus the
light rays on the retina. This increase in refractive power in
caused by increasing the curvature (and hence the dioptric
strength) of the lens. When very young children focus on an
extremely close object they can increase their dioptric strength
from 18 to nearly 32 diopters. This represents an accommodation
of 14 diopters in adjusting from distant to near vision.
Notice that accommodation is greatest in the very young child
(about 14 diopters), decreases to about 11.5 in the young adult,
and is not much better than 2 or 3 in the elderly. Accordingly,
the near point increases with age. It is typically about 12 cm
in the young adult and often reaches 100 cm in the elderly.
Thus we see the familiar pattern of the aging person holding
reading material farther and farther away from the eyes in
order to be able to focus on it. Of course since 100 cm is
beyond the reach of the arms, reading glasses are often
required.
Emmetropia, Hypermetropia, and Myopia
In normal vision
(emmetropia)
parallel light rays from a viewed object are brought to a focus
exactly on the retina. The individual perceives the image as
sharp, clear, and in focus. However, if the refractive surfaces
of the eye can't focus the parallel rays on the retina, the
image is blurred and corrective glasses or contact lenses are
required. If insufficient light bending occurs, parallel rays
aren't sufficiently refracted to be brought to a focus on the
retina. This condition is called
hypermetropia
and the individual is said to be far-sighted. That is, he can
focus well on distant objects which don't require much light
bending, but can't focus well on objects up close. On the other
hand, if the refractive power of the eye is so great as to focus
parallel rays in front of the retina, the image is also blurred
and the condition is called
myopia
(near-sightedness).
Neural Control
and Accommodation of the Lens
The ciliary muscle is innervated by both the somatic and
autonomic nervous systems. Through the former, we are able to
voluntarily change the focus from near to far vision by altering
the thickness and curvature of the lens. The geometric
orientation of the ciliary muscle is such that when it is
relaxed, the suspensory ligament is taut and the lens is pulled
flat, setting it for distant vision (Fig-2). Since the eyes
are set for distant vision most of the time, it follows that the
ciliary muscle is usually relaxed. Contraction causes the suspensory ligament to become less taut and allows the lens to
assume the more spherical (and hence more powerful) shape.
The shape of the lens is also automatically adjusted as the gaze
shifts between near and far vision. The autonomic nervous
system regulates automatic adjustments. Parasympathetic
stimulation contracts the ciliary muscle and thereby increases
the refractive power of the lens. Sympathetic stimulation
appears to relax the muscle, decreasing the strength of the
lens (Fig-5).
Depth of Focus
and Accommodation of the Pupil
Further examination of Fig-5 will show that pupil diameter
is also under autonomic control. GVE fibers of the oculomotor
nerve (III) supply parasympathetic innervation to the sphincter
muscle of the iris. while sympathetic fibers innervate the
radial muscles. Contraction of the former causes the pupils to
constrict, while contraction of the latter produces pupillary
dilation.
The amount of ambient light to a large extent determines the
size of the pupillary aperture. In low-light situations
the pupils dilate to allow the available light to reach the
retina. In bright daylight the pupils are constricted in
order to limit the amount of light entering the eyes. The pupils
also automatically constrict when viewing objects at very close
range and dilate when the gaze shifts to distant vision. The
depth of focus
is greatest in bright light when the pupillary aperture is small
(i.e., 2 mm). On the other hand, it is minimum in dim light when
the aperture is large (i.e., 8 mm). All things being equal, a
large depth of focus means that a viewed object can move back
and forth a slight distance without going out of focus. On the
other hand, if the depth of focus is very small, even the
slightest movement will put the object out of focus (Fig-6). |
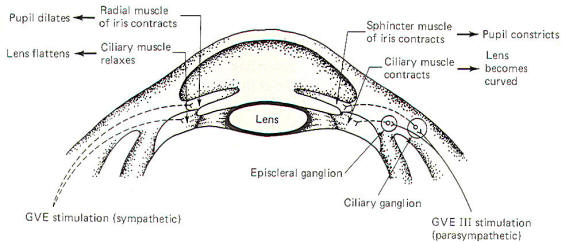 |
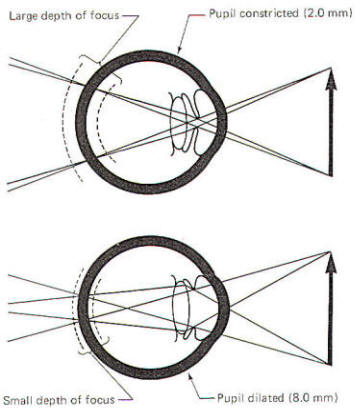 |
Fig-5 |
Fig-6 |
OPTIC REFLEXES
Pupillary Light
Reflex
This is the well-known response in which the pupils constrict in
bright light. The reflex arc employed in this response is
illustrated in Fig-7. If
an equal amount of light shines into both eyes, the degree of
constriction is generally equal. However, if the light is
directed primarily into one eye (i.e., with a flashlight), the
pupil of that eye greatly constricts
(direct reflex)
while the pupil of the other eye shows a much smaller degree of
constriction
(consensual reflex).
Notice that some of the fibers of the optic tracts pass to the
colliculi of the upper midbrain rather than continuing on to the
lateral geniculate bodies. These fibers project to both the
ipsilateral and contralateral superior colliculi. Short neurons
project from the colliculi to the Edinger-Westphal nucleus (an
accessory nucleus of III)
in the midbrain, which serves as the origin of preganglionic
parasympathetic fibers (GVE) of the oculomotor nerves (III). The
GVE III
fibers, in turn, project to the ciliary ganglia from which
postganglionic fibers innervate the sphincter muscles of the
iris. If the light is directed evenly into both eyes, the
pupillary change is uniform. However, if it is directed
primarily into one eye, the neural firing is "weighted" toward
that side and the greatest constriction is observed on that
side.
Accommodation Reflexes As an Object Is Brought Closer to the
Eyes
When an object is brought closer to the eyes we must make visual
adjustments (accommodations) in order to keep it in sharp focus.
These accommodations include (l) convergence of the eyes, (2)
thickening of the lenses, and (3) pupillary constriction.
Convergence is necessary in order to keep the viewed object
lined up with the visual axis of each eye. This keeps the object
focused on the fovea for maximum visual acuity. As a viewed
object is brought closer, light rays from any single point
source on the object become less parallel, and the refractive
power of the lens must be increased in order to focus the image
on the retina. The lens accomplishes this by becoming thicker
and more spherical, thereby increasing its refractive power.
Finally, in order to increase the depth of focus (always a
problem at short distances), the pupils constrict. It should be
pointed out that one can consciously "override" the
accommodation reflexes and prevent their occurrence. However,
lacking such conscious effort, they proceed automatically.
Convergence of the eyes is brought about by the following reflex
pathway.
GSE fibers of the oculomotor nerves (III) in the nucleus of
III
in the midbrain tegmentum become stimulated via an undefined
route from the visual cortex. These fibers then project to the
medial rectus muscles of the eyeballs, causing them to contract.
This produces an inward turning of the eyes, keeping the viewed
object focused on the fovea for maximum visual acuity. The
oculomotor nerves also innervate the superior oblique and
superior and inferior rectus muscles of the eyes as well. Thus,
by selective stimulation of the appropriate GSE III
fibers, specific muscles can be activated, causing appropriate
degrees and angles of convergence.
Thickening of the lens and constriction of the pupils are both
produced through reflex pathways involving the parasympathetic (GVE)
fibers of the oculomotor nerve. The pathway for pupillary
constriction is identical to that of the pupillary light reflex,
starting from the Edinger-Westphal nucleus. However, it appears
likely that signals are relayed to this nucleus via undefined
routes from the visual cortex in the occipital lobe. Some of the
GVE III
fibers which enter the ciliary ganglia pass right through
without synapsing to enter the episcleral ganglia (Fig-5).
Postganglionic fibers project from here to the ciliary muscle,
causing it to contract, thereby thickening the lens and
increasing its refractive power.
|
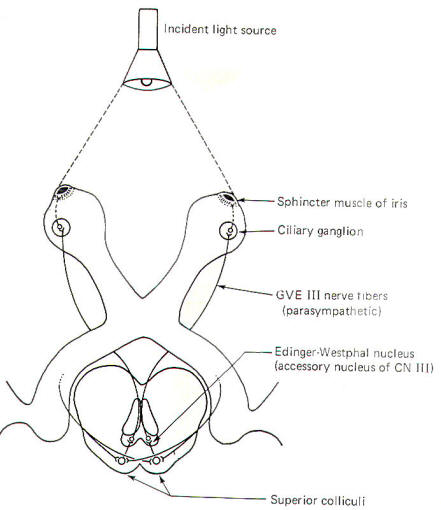 |
Fig-7 |
THE CONSCIOUS
VISUAL PATHWAY
We previously mentioned that impulses on the optic nerve give
rise to both conscious visual images and a variety of purposeful
optic reflexes. The conscious visual pathway, which we will
examine now, is illustrated in Fig-8. In order to
give rise to a conscious image, impulses generated by light
stimulation of the retina must be transmitted to areas 17, 18,
and 19 of the optic lobe. Area 17 is the primary visual area and
initially receives the signals from the optic radiations.
However, the visual association area (areas 18 and 19) helps to
"make sense" out of the signals reaching area 17.
The retina of each eye is divided into a medial half, the
nasal retina, and a lateral half is called the temporal
retina. Optic nerve fibers from the nasal retina of each eye
cross over in the optic chiasm and terminate in the
lateral geniculate body on the contralateral side (Fig-8).
Those from the temporal retina do not cross over in the chiasm
but continue instead on the same side, terminating in the
ipsilateral lateral geniculate body. The optic nerve is
composed of that portion of nerve fibers between the eye and
the optic chiasm. The continuation of the fibers from the optic
chiasm to the lateral geniculate bodies are collectively called
the optic tracts. Thus the optic nerves contain fibers
from only one eye, while the optic tracts are composed of fibers
from both eyes. The optic chiasm lies just anterior to the
pituitary gland.
Fibers carrying visual signals from each lateral geniculate body
project posteriorly as the optic radiations, terminating
in the visual cortex of each occipital lobe. Some of these
fibers terminate in the cuneus above the calcarine
fissure, and some terminate below it in the lingula.
Figure-9 illustrates the conscious visual pathway when a
single quadrant of the retina is stimulated. Notice that light
from the left visual field of each eye stimulates the nasal
retina of the left eye and the temporal retina of the right eye.
Further, light from the lower left visual field of both eyes
stimulates the upper right quadrants of the nasal retina of the
left eye and the temporal retina of the right eye. A little
thoughtful examination of Fig-9 will enable you to
appreciate the relationships between a point source of light in
the visual field and the retinal quadrant which it stimulates.
The image focused on each retinal quadrant is represented on a
specific area of the visual cortex. The upper right quadrant of
each eye projects to the right cuneus while the upper left
quadrant of each eye projects to the left cuneus. Similarly, the
lower right quadrant of each eye projects to the right lingula
while the lower left quadrants project to the left lingula. Thus
the fibers of the optic radiation are spatially oriented with the
more superior half of the radiation projecting to the cuneus,
while the inferior half projects to the lingula. |
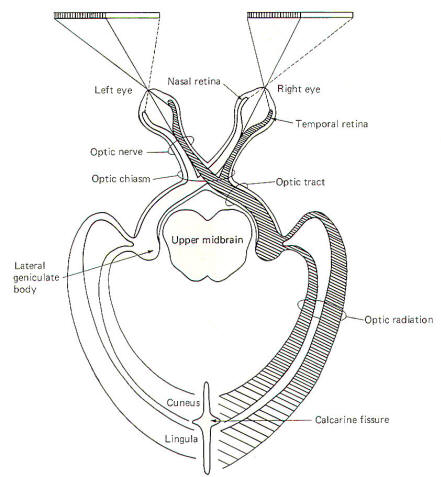 |
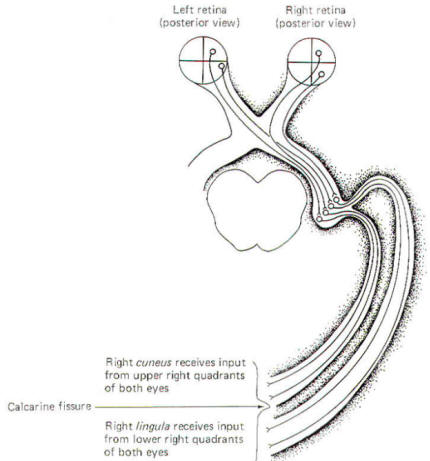 |
Fig-8 |
Fig-9 |
Injury to the
Conscious Visual Pathway
Figure-10 illustrates the defects in viewing the visual field
associated with lesions to several specific locations in the
conscious visual pathway. A complete lesion in a single optic
nerve causes total anopsia (blindness) in that eye. Vision in
the opposite eye is unaffected. An interior-posterior lesion
through the middle of the optic chiasm interrupts only the
crossing fibers (those from the nasal retinae) while leaving
those from the temporal retinae intact. Now because the nasal
retinae are stimulated by light from the lateral (temporal)
visual fields, the visual field loss is called heteronymous
bitemporal hemianopia. Hemianopia means that the loss is to
one-half of the visual field. Heteronymous means that the loss
is to different visual fields for each eye. However, a lesion to
the optic tract eliminates visual signals from the nasal retina
of the contralateral eye as well as the temporal retina of the
ipsilateral eye. This condition is also described as hemianopia
since one-half of the visual field is eliminated from each eye,
However. the hemianopia is homonymous as the loss is to
the same visual field of each eye. Lesions of the optic
radiation might cause either hemianopia (entire radiation
affected) or loss in only one quadrant if cuneal or
lingular radiation fibers are selectively damaged.
Of course partial loss can occur if the damage to
the superior or inferior part of the radiation is
only partial. |
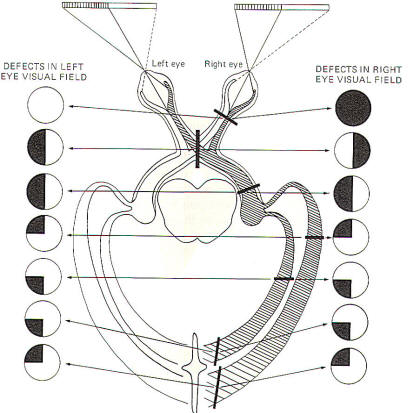 |
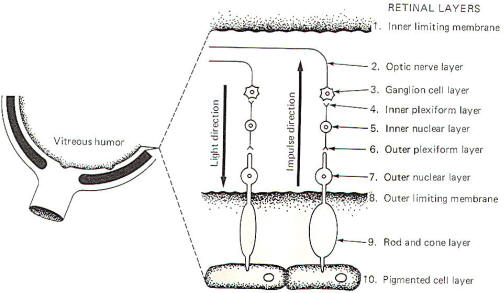 |
Fig-10 |
Fig-11 |
THE RETINA:
THE LIGHT-SENSITIVE PORTION OF THE EYE
Photons of light entering the eye must reach the light-sensitive
rods and cones of the retina before neural signals are generated
in the visual system. Figure11
diagrammatically illustrates the 10 characteristic layers of the
retina. Notice that the direction of light is opposite to the
direction of the generated impulses.
Light first reaches the retina by passing through the inner
limiting membrane. The impinging light rays next encounter the
optic nerve layer which is composed of the fibers of the
ganglion cells (optic nerve cells), whose cell bodies comprise
the ganglion cell layer. Deeper bipolar neurons synapse with
these cells in large dendritic arborizations forming the inner
plexiform layer. The peripheral processes of these bipolar
neurons receive synaptic input from the rods and cones in the
outer plexiform layer. The cell body region of the bipolar
neurons makes up the inner nuclear layer. The outer nuclear
layer is the region of rod and cone cell bodies. The outer
limiting membrane separates the outer nuclear layer from the
enlarged photosensitive ends of the rods and cones in the rod
and cone layer. Finally, the rods and cones are in close
functional contact with pigmented epithelial cells, which
comprise the tenth layer of the retina.
Photoreceptor
Stimulation and Impulse Production
Light passing through the retinal layers stimulates the enlarged
photosensitive portion of the rods and cones before being
absorbed by the pigmented epithelium. Once activated, the rods
and cones stimulate the bipolar neurons, which in turn excite
ganglion cells, producing impulses in the optic nerve. It is
likely that a receptor potential is generated on the
photoreceptor cell membrane, which then generates impulses in
the bipolar and ganglion cells. No impulses have actually been
recorded in the rods and cones themselves.
The absorption of light by the pigmented epithelium is important
for visual acuity as it prevents reflected light from
stimulating rods and cones in other areas of the retina. By
minimizing reflected light, the optical quality of the image
detected by the array of photoreceptors in the retina is
improved. The fovea is capable of generating higher visual
acuity than any other part of the retina partly because it has
more pigment in its tenth layer. Also, the cones in the fovea
are more slender than elsewhere in the retina and thus produce a
finer "grain" to their image.
The visual signals generated in the rods and cones converge
considerably upon reaching the ganglion cells. Figure-11
gives the impression that each ganglion cell is in direct
contact with a single bipolar neuron which is stimulated by a
single rod or cone cell. Actually there are many more rods and
cones than bipolar neurons, and many more bipolar neurons than
ganglion cells. Each retina contains approximately 125 million
rods and 5.5 million cones. Thus an average of 140 rods and 6
cones feed visual information into a single ganglion cell. This
considerable convergence of the visual signal may possibly add
"sharpness" to the visual image.
RHODOPSIN: THE
PHOTOSENSITIVE CHEMICAL IN RODS AND CONES
Both rods and cones contain a photosensitive pigment, rhodopsin,
which decomposes on exposure to light, releasing sufficient
energy to establish a receptor potential on the photoreceptor
cell membrane. Rods and cones appear to stimulate the bipolar
neurons through chemical transmission. Whether the central state
of the bipolar neurons is raised above the excitation threshold
is probably a function of the quantity of chemical transmitter
released, which itself is probably a function of the magnitude
of the receptor potential developed on the photoreceptor.
Rods and cones have different characteristics. Rods are usually
narrower (4 to 5
µm)
than cones (5 to 8
µm).
The photochemicals in the two kinds of photoreceptors are
slightly different. Their functional capabilities are also
different. Cones function best at high intensities of light such
as that associated with daylight vision. Further, they are
responsible for color vision and are characterized by high
visual acuity, Rods, on the other hand, have higher sensitivity
and are more suited for vision at night, when light intensity is
low. They don't mediate color vision nor are they able to
resolve fine detail. Most of the research on photoreceptor cells
has been done on rods because of their relatively great number.
Consequently, most of the functional information we have about
the chemistry of rhodopsin has been obtained from rod studies.
Nevertheless, there is evidence that cones probably function in
a similar manner.
Rhodopsin is a complex chemical with a protein portion complexed
with a caratinoid pigment, cis-retinine. The difference between
rod rhodopsin and cone rhodopsin is in the protein portion. Rod
rhodopsin (scotopsin) differs from cone rhodopsin (photopsin) by
the number, type, and sequence of its amino acids. In
either case, exposure of rhodopsin to light causes it to
decompose with the release of sufficient energy to establish a
receptor potential on the cell membrane.
The Rhodopsin
Cycle
When rhodopsin is decomposed by light, releasing energy, the
breakdown products of this decomposition subsequently recombine
to synthesize more rhodopsin. The sequence is called the
rhodopsin cycle
(Fig-12).
Rhodopsin is a stable molecule. However, once exposed to light,
it undergoes a configurational change becoming first
lumirhodopsin and then
metarhodopsin.
meta-Rhodopsin
is quite unstable and quickly decomposes to
trans-retinine
and scotopsin. This latter decomposition is accompanied by the
release of sufficient energy to produce a receptor potential on
the cell membrane. Most of the
trans-retinine
undergoes enzymatically catalyzed isomerization, becoming
cis-retinine. Once formed, cis-retinine combines with scotopsin,
reforming rhodopsin.
In
dim light it is important that sufficient amounts of rhodopsin
are available for decomposition so that the rods are maximally
sensitive to any light which is present. On the other hand, when
plenty of light is available, much of the trans-retinine is
shunted into the pigmented epithelial cells which are in close
contact with the photoreceptor cells (Fig-12). Here it
undergoes conversion to
trans-vitamin
A. Of course, shunting
trans-retinine
into the pigment cells decreases the amount of rhodopsin
available for decomposition by light, thus decreasing the
sensitivity of the retina. This is certainly a desirable feature
when abundant light is available.
trans-Vitamin A in the pigmented epithelial cells is in chemical
equilibrium with its isomer, cis-vitamin A. The
trans-retinine-trans-vitamin
A and cisretinine-cis-vitamin
A interconversions are oxidation-reduction reactions. A constant
supply of vitamin A is made available to the epithelial cells by
capillaries of the choroid plexus, the vascular layer between
the retina and the sclera (Fig-1).
Light Adaptation
A person in the dark for up to 30 min is said to be
dark-adapted.
That is, retinal sensitivity has increased to a sufficiently
high level so that the eyes are sensitive to whatever minimal
light is available. When the person subsequently moves into a
well-lighted environment, everything is initially very bright
due to the high sensitivity of the retina, and visual acuity is
initially quite poor. So much rhodopsin is being decomposed that
a "flash" of light rather than any fine detail is experienced.
After a few seconds to a minute, the eyes adapt to the light and
the retinal sensitivity is decreased. This is
light adaptation.
It is caused by the conversion of retinine to vitamin A and its
subsequent storage in the pigment cells. Since the rate-limiting
step for the reformation of rhodopsin is the availability of retinine, this effectively reduces the stores of rhodopsin in
the photoreceptors and decreases their sensitivity.
|
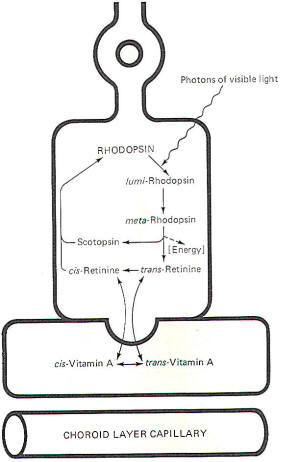 |
Fig-12: The Rhodopsin cycle. |
Dark Adaptation
A light-adapted person has low retinal sensitivity. That is, rod
and cone rhodopsin stores have been reduced to a relatively low
level by the conversion of retinine to vitamin A and its
subsequent storage in the pigment cells. Now when the
light-adapted person suddenly enters a very dark room, the
available light is drastically reduced and it becomes necessary
to increase the sensitivity of the retina in order to see. This
process is called
dark adaptation.
Dark adaptation is a slower process than light adaptation. The
adaptation occurs as stored vitamin A is converted to retinine,
which immediately complexes with scotopsin to reform more
rhodopsin. This obviously increases the rhodopsin stores and
hence the sensitivity of the retina.
Insufficient dietary vitamin A intake can produce chronic low
retinal sensitivity since it is a necessary precursor for rhodopsin production. The effects are more severe at night when
high retinal sensitivity is necessary. The condition is called
night blindness
and is directly related to the lack of vitamin A. It takes weeks
for a dietary deficiency to bring on symptoms, however, as the
liver is capable of storing large amounts of vitamin A.
Figure-13 illustrates the increase in retinal sensitivity as
dark adaptation progresses. The bimodal characteristic of the
curve is due to the differences in rod and cone adaptability.
Cones adapt more quickly than rods. That is, they resynthesize
retinine from vitamin A at a greater rate. The initial small
increase in sensitivity upon entering a dark room is due to
activity in the cones, which start to adapt immediately.
However, because of the relatively few cones compared to rods,
the overall increase in retinal sensitivity due to cone
adaptation is quite small. On the other hand, while rods adapt
more slowly, they contribute much more to the overall increase
in retinal sensitivity because of their relatively great
numbers. Three-quarters of an hour is often required for full
adaptation to the dark. |
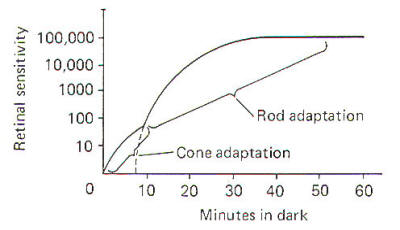 |
Fig-13: |
Color Vision
While only one type of rod is found in the vertebrate retina,
there are three types of cones. Each has its own color-sensitive
pigment, making cones the photoreceptors responsible for color
vision. The difference in the pigments of each type of cone
probably lies in the opsin (protein) portion of the cone
rhodopsin. Each type of cone responds maximally to a different
wavelength of light. These three types are illustrated in Fig-14.
Blue cones
respond maximally to light of 430 nm (nanometers) wavelength,
green cones
to 535 nm, and
red cones
to 575 nm. Figure-14 is a spectral sensitivity curve for each
of the three types of cones. Notice that while each cone is
maximally sensitive to a specific wavelength, it nevertheless
will respond (though to a lesser extent) to other wavelengths as
well. Notice also that the response curves of the three types of
cones significantly overlap.
The Young-Helmholtz theory of color vision states, in part, that
the ratio of the relative responses of each different type of
cone stimulated determines the color we see.
If
monochromatic light of 575-nm wavelength is presented to the eye
and is focused on the retina, the response of the cone types in
this area of the retina will be different. Examination of Fig-14 shows that the red cones will be maximally stimulated, the
green cones will be stimulated to 50 percent maximum, and the
blue cones will not be stimulated at all. Therefore the response
ratio of the cones (red:green:blue) is 100:50:0. The brain
decodes this signal and interprets it as the color yellow.
Similarly, a monochromatic light of 535 nm would produce the
ratio 65: 100:0 and be interpreted as green. A light of 502 nm
would give rise to the response ratio 30:60:30 and would be seen
as blue-green, and so on.
|
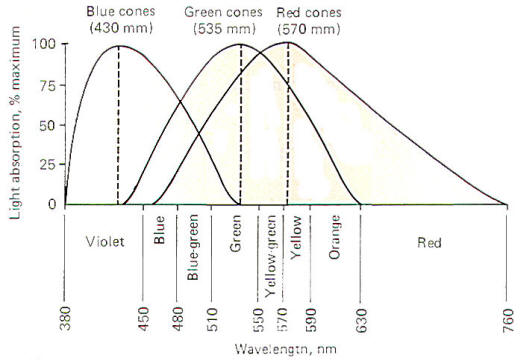 |
Fig-14: The
spectral sensitivity of the the types of cones in
the retina. |
|
 |
 |
Our brain is a mystery and to understand it, you
need to be a neurosurgeon, neuroanatomist and neurophysiologist.
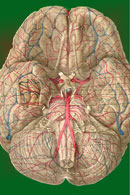
neurosurgery.tv

Please visit this site, where daily neurosurgical activities are going
on.
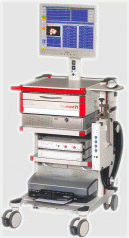
Inomed ISIS IOM System
|
|
|
|